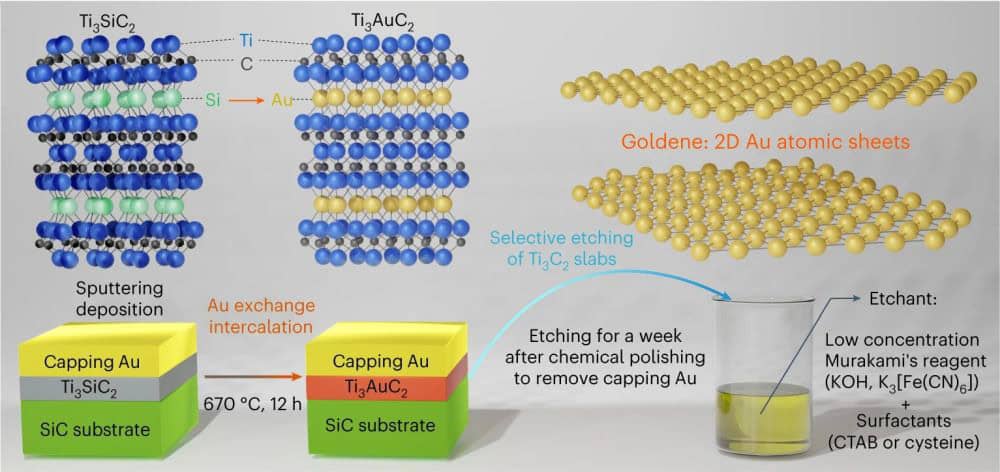
[Image above] Schematic illustration of the preparation of goldene. Credit: Kashiwaya et al., Nature Synthesis (CC BY 4.0)
By Laurel Sheppard
Since the discovery of graphene in 2004, researchers have successfully created single-atom-thick sheets out of several materials, including boron and phosphorus. However, to date, some materials have resisted single-layer formation, such as gold.
Gold is of interest to materials researchers because typically it is conducting. However, “If you make a material extremely thin, something extraordinary happens—as with graphene. The same thing happens with gold…if single-atom-layer thick, the gold can become a semiconductor instead,” explains Shun Kashiwaya, researcher in the Materials Design Division at Linköping University, Sweden, in a recent press release.
This switch from being a conducting to semiconducting material, in addition to other behavioral changes, means single-atom-thick gold may be useful in applications ranging from carbon dioxide conversion to hydrogen production to water purification, among others.
Unfortunately, gold atoms have a strong thermodynamic tendency to lump together, which complicates the production of single-atom-thick sheets. As such, previous work has only achieved gold monolayers confined on or inside templates, while the thinnest reported free-standing gold sheet is two atoms thick, created via a wet-chemical route using methyl orange.
In April 2024, Kashiwaya and his colleagues at Linköping University announced they had finally developed a process for synthesizing true goldene, i.e., single-atom-thick gold sheets. This method, which they described in an open-access paper, mirrors the process used to create the well-known family of 2D transition metal carbides and nitrides called MXenes.
MXenes are a derivative of the well-known class of bulk layered ternary carbides and nitrides called MAX phases, which are composed of a transition metal (M), an A-group element (A), and carbon or nitrogen atoms (X). MXenes are often produced by etching away the A layers from MAX phases, leaving Mn+1Xn layers. This process works because most A elements are weakly bonded to the neighboring M elements, while the M–X bonds are relatively strong.
In the new gold study, the Linköping researchers took the MAX phase Ti3SiC2 and swapped the silicon atoms for gold. As explained in a press release, they initially discovered this substitution process several years before, but they did not know how to extract the gold afterward.
Now, though, they discovered they could borrow from a century-old Japanese knife production method, which uses an alkaline potassium ferricyanide solution called Murakami’s reagent to remove carbon residue and change the color of steel. They combined this reagent with a surfactant, either cetrimonium bromide or cysteine, to etch away Ti3C2 from the nanolaminated Ti3AuC2, thus leaving free-standing single-atom-thick gold sheets.
Notably, this process was conducted in the dark because cyanide develops when the reaction takes place under light and subsequently dissolves the gold, according to the researchers.
Adding a surfactant improved the stability of the sheets from curling up and forming blobs near the etched-free edges. The curling and blob formation was attributed to external factors related to the initial substitution of gold for silicon in the Ti3SiC2 MAX phase as well as interactions during the subsequent etching process.
The outcome of the etching process was dependent on the concentration of the reagent and the etching time. Gold layers became thicker as the etching time increased. On the other hand, a lower concentration led to better yield and quality of the resulting gold layers.
The optimum concentration for the etching process was between 0.2 and 1%. As the researchers explained, “The 0.1% concentration resulted in complete decomposition of 2D Au [gold] layers into 3D spherical nanoparticles, due to the lower concentration needing a much longer etching duration. The etchants would gradually attack the freed Au layers initially stabilized by surfactants as the radical nascent oxygen produced from the etchants eventually attacks the surfactants.”
To better understand the factors affecting the gold monolayer’s structural stability, the researchers conducted ab initio molecular dynamic simulations. These simulations showed that free-standing 2D layers of gold coalesced in the presence of silicon impurities or if brought to within 7 angstroms distance of each other. When the separation distance increased to 10, 12, and 14 angstroms, the gold—gold interlayer interaction significantly decreased, allowing the surfactants to stabilize the gold sheets against coalescence so they are preserved.
Electron microscopy also showed that interlayer distance between gold layers within the MAX lattice plays a critical role in preparing goldene. For example, the microscopy analysis revealed that a roughly 9% lattice contraction takes place in the gold monolayers compared to bulk gold. The estimated gold–gold spacing was close to the calculated gold–gold bond length (2.62 vs 2.735 angstroms, respectively) for optimized, tightly packed 2D gold. The shorter spacing was attributed to stronger in-plane bonding.
The Linköping researchers’ process has the advantages of being simple, scalable, and free of hydrofluoric acid, as well as minimal use of gold. In addition, the area of the goldene sheet could possibly be expanded by means of etching and surfactant optimization.
“The template SiC(0001) wafer size that sets the area limit of growing epitaxial Ti3SiC2 for subsequent Au intercalation is currently 200 mm, which would give an ample allowance for practical goldene application,” the researchers write.
The press release notes that in future work, the researchers will investigate whether a similar process can be used to produce single-atom-thick sheets of other noble metals.
The open-access paper, published in Nature Synthesis, is “Synthesis of goldene comprising single-atom layer gold” (DOI: 10.1038/s44160-024-00518-4).